Breaking Barriers in Quantum Fidelity: MIT’s Groundbreaking Innovation with Fluxonium Qubits
- Dr. Shahid Masood
- Jan 22
- 5 min read

Quantum computing is not merely a futuristic vision anymore. As researchers make new strides in developing quantum hardware and algorithms, the goal of building a practical, fault-tolerant quantum computer is becoming increasingly attainable. The recent breakthrough at MIT, where researchers achieved a remarkable 99.998% fidelity using fluxonium qubits, has sparked a new wave of excitement in the quantum computing community. This achievement is a significant milestone on the road to developing scalable and practical quantum systems capable of solving real-world, complex problems that classical computers struggle to address.
The Challenges of Quantum Fidelity: Why It Matters
At the heart of any quantum computing system lies the qubit, the fundamental unit of quantum information. Qubits, unlike classical bits, can exist in multiple states simultaneously thanks to the principles of superposition. However, qubits are highly sensitive to noise and imperfections. Even minor fluctuations in their environment can introduce errors that disrupt the quantum state, compromising the integrity of computations.
Quantum Fidelity is a measure of the accuracy with which a quantum operation is performed on a qubit. Achieving high fidelity is essential because errors in quantum operations accumulate quickly, reducing the reliability of quantum algorithms. The better the fidelity, the less likely the quantum system is to experience errors, allowing it to execute complex algorithms more accurately.
The 99.998% fidelity achieved by MIT's research team represents a dramatic improvement in qubit performance. Prior to this, even the best superconducting qubits had fidelities in the range of 99%, with many practical quantum systems struggling to push beyond 98%. This significant leap in fidelity takes us closer to the realization of a quantum computer that can perform practical tasks with near-perfect precision.
What Makes Fluxonium Qubits Special?
Fluxonium qubits, which were central to MIT’s achievement, are part of the superconducting qubit family, a leading approach for building quantum processors. However, fluxonium qubits distinguish themselves from other types due to their exceptional properties, which make them more resistant to noise and other errors.
A fluxonium qubit consists of a superconducting loop interrupted by a Josephson junction, which creates a well-defined quantum state. Unlike traditional superconducting qubits, fluxonium qubits are designed to have a lower energy gap, resulting in longer coherence times. This increased coherence time allows fluxonium qubits to maintain their quantum state longer, which is essential for reducing errors during quantum operations.
The superinductor in the fluxonium qubit is a key element that provides this noise resistance. The superinductor is a type of inductor that behaves as if it has an extremely large inductance, which suppresses energy loss and improves the stability of the qubit’s quantum state.
However, fluxonium qubits have a downside: their lower operational frequency means that quantum gates take longer to perform compared to higher-frequency qubits. This trade-off between coherence and speed has been a challenge for scaling fluxonium qubits for practical use, especially in algorithms requiring rapid quantum gate operations.
Achieving 99.998% Fidelity: Overcoming Counter-Rotating Errors
One of the significant obstacles that MIT researchers faced in achieving such high fidelity was the problem of counter-rotating errors. These errors arise when qubits are manipulated using electromagnetic pulses that induce oscillations in the qubit state. These oscillations, when applied too quickly, can result in discrepancies between the intended and actual qubit states.
In the case of fluxonium qubits, these counter-rotating errors became more prominent due to the longer gate times associated with their lower operating frequency. To overcome this challenge, the researchers developed two innovative control techniques:
Commensurate Pulses: By applying control pulses at specific intervals synchronized with the qubit's natural frequency, the MIT team was able to reduce the randomness of counter-rotating errors. These well-timed pulses make the errors predictable and correctable, enabling higher fidelity.
Synthetic Circularly Polarized Microwaves: The team also utilized synthetic circularly polarized microwaves to manipulate the quantum state of the qubit. Circularly polarized microwaves are commonly used in optical systems but have now been adapted to control superconducting qubits, offering precise and stable control over the qubit's state.
These two techniques allowed the MIT team to mitigate counter-rotating errors effectively and achieve the highest fidelity for fluxonium qubits to date.
Fidelity Comparison of Quantum Systems
Quantum System | Fidelity Achieved | Key Features |
MIT Fluxonium Qubit | 99.998% | Superconducting qubit with longer coherence time |
Google’s Sycamore Processor | 99.97% | Superconducting qubit, notable for quantum supremacy |
IBM Qiskit | 99.5% | Superconducting qubits, general-purpose quantum computer |
IonQ Trapped Ions | 99.99% | High-fidelity ion-trapped qubits |
Rigetti Aspen-9 | 99.8% | Superconducting qubits optimized for quantum applications |
The data above highlights MIT’s new record, surpassing the previously best-performing systems in superconducting qubits, with a fidelity closer to the ion-trapped qubit systems. This leap sets a new benchmark for the field.
Fluxonium Qubits and Their Impact on Quantum Computing
The remarkable performance of fluxonium qubits underscores their potential for use in both quantum algorithms and fundamental physics research. The coherence time of fluxonium qubits is far superior to that of many other superconducting qubits, making them well-suited for long-duration quantum operations. The ability to mitigate errors while maintaining high coherence means that fluxonium qubits can now compete with ion-trapped qubits, which have long been regarded as the gold standard for quantum systems.
The table below provides an overview of the key advantages and challenges associated with fluxonium qubits compared to other superconducting qubits.
Feature | Fluxonium Qubits | Other Superconducting Qubits | Ion-Trapped Qubits |
Coherence Time | Long | Moderate | Long |
Operational Frequency | Low | High | Moderate |
Error Resistance | High | Moderate | High |
Gate Speed | Slow | Fast | Moderate |
Scalability | Moderate | High | Low |
As the table shows, fluxonium qubits offer excellent noise resistance and long coherence times, but their slower gate speeds present challenges in scaling up for fast-paced algorithms. However, with the improvements made in mitigating counter-rotating errors, these challenges are becoming increasingly manageable.
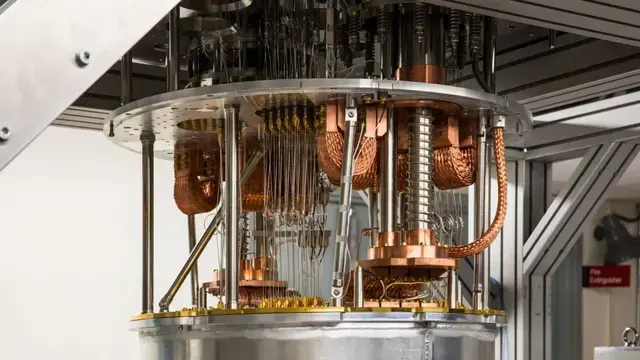
Fault-Tolerant Quantum Computing: A Step Closer
The issue of fault-tolerant quantum computing remains one of the most critical barriers in scaling up quantum systems. Fault tolerance is the ability of a quantum computer to continue functioning correctly despite errors in the qubits. Achieving high fidelity is a key step toward fault tolerance because errors can accumulate over time and cause a quantum algorithm to fail.
The MIT team's breakthrough is a significant advancement in this regard. By developing methods to correct counter-rotating errors efficiently, the researchers have made quantum systems more robust and reliable. These techniques not only improve the performance of fluxonium qubits but can also be applied to other types of quantum qubits, providing a universal solution to one of the most challenging aspects of quantum computing.
The Road Ahead for Quantum Computing
While MIT’s achievement is groundbreaking, quantum computing remains in its infancy. Challenges such as scalability and error correction still need to be addressed before large-scale, fault-tolerant quantum computers can be built. However, the progress made with fluxonium qubits demonstrates the potential of superconducting qubits and the power of new control techniques to overcome long-standing limitations.
As quantum hardware improves, it is expected that quantum computers will eventually become indispensable tools for solving complex problems in areas such as:
Cryptography: Quantum computers have the potential to break existing encryption systems and lead to the development of new, quantum-safe encryption methods.
Drug Discovery: Quantum computers could simulate molecular interactions with unprecedented precision, accelerating the process of developing new drugs.
Optimization: Quantum optimization algorithms could revolutionize industries such as logistics, finance, and energy by solving complex optimization problems at scale.
A New Era for Quantum Computing
MIT’s achievement in demonstrating 99.998% fidelity with fluxonium qubits is a major milestone in the journey toward practical quantum computing. This breakthrough not only showcases the power of superconducting qubits but also brings us closer to building quantum systems capable of solving real-world problems. As researchers continue to refine quantum hardware and algorithms, the future of quantum computing looks increasingly bright.
To stay ahead in the rapidly evolving field of quantum computing, follow Dr. Shahid Masood and the expert team at 1950.ai for continuous updates on groundbreaking developments in artificial intelligence, quantum computing, and other cutting-edge technologies. Stay informed and learn more about the future of tech innovations.
More groundbreaking attempts like this one can help us to trade off better between speed, accuracy and fault tolerance.